Techniques
As a leader in photonics innovation CAPPA uses a variety of techniques to conduct their research. It describes the basic principles behind the operation of the experimental setups and the interpretation of the data. Some of the investigative techniques CAPPA have include; time – resolved photoluminescence, pump probe spectroscopy, frequency resolved electro – absorption gating (FREAG) and mode – locked lasers.
Time-Resolved Photoluminescence
Time Resolved Photo-Luminescence (TRPL) is an experimental technique that provides the spectral and temporal evolution of the emission of a sample following its illumination by a short pulse of light. More precisely, the short pulse of light generates electron-hole pairs that decay to lower energy levels of the sample. These electron-hole pairs can subsequently recombine and emit light. The emitted light is composed of a set of wavelengths corresponding to transition energies of the sample and, as a result, the measurement of the optical spectrum as a function of time provides a means to measure the transition energies and their lifetimes. Since these decay times are on the order of picoseconds or nanoseconds, and the intensity of light emitted can be very weak, a conventional spectrum analyser cannot provide the resolution required. Instead, it is necessary to use a device known as a streak camera.
The streak camera is an ultra-high-speed detector that captures light emission phenomena occurring in extremely short time periods. The light pulse to be measured is projected onto the slit and is focused by a lens into an optical image on the photocathode, able to cover a wavelength range between 300 nm and 1500 nm. Here, the photons are converted into a stream of electrons proportional to the intensity of the incident light. As the electron stream created from the light pulse passes between a pair of sweep electrodes, a time-varying voltage is applied to the electrodes, resulting in a high-speed sweep. This means that the early part of the pulse is deflected less than the later part of the pulse, so that different parts of the pulse strike the micro channel plate (MCP) at different positions. Thus, the temporal structure of the pulse is converted into a spatial distribution, or ‘streak’, pattern. As the electrons pass the MCP, they are multiplied several thousands of times and are then bombarded against the phosphor screen, where they are converted back into light. The fluorescence image corresponding to the early part of the incident light pulse is positioned at the top of the phosphor screen, with later parts positioned in descending order; in other words, the axis in the perpendicular direction on the phosphor screen serves as the temporal axis. The brightness of the fluorescence image is proportional to the intensity of the corresponding incident light pulses and the position in the horizontal direction on the phosphor screen corresponds to the wavelength of the incident light.
To perform TRPL experiments, the sample under investigation is mounted on a cold finger in the vacuum cell of a closed cycle helium cryostat (operating from 7 K to 300 K), and is illuminated with very fast (picosecond or femtosecond) laser pulses. The optical response is then collected by a microscope objective and is sent to the entrance slit of an imaging spectrograph, directly connected with the input optic of a streak camera.
The typical spectrum collected by a TRPL experiment is called a streak image. It depicts the intensity of the optical response of the sample (colour scale) as a function of the wavelength (horizontal axis) and the time (vertical axis). One such example concerns the emission dynamics of an InGaAs/GaAs quantum well (QW) at 8 K. A 780 nm laser pulse excites the InGaAs QW above the band gap of the material (~890 nm at this temperature), generating photo-carriers in the GaAs barrier. From here, they flow into the well, and recombine to emit light. As the number of carriers is depleted, the intensity of the emitted light decays. The carrier dynamics occurring during these processes provides not only information about the position of the peak emission, as in a normal PL experiment, but also the lifetime of the exactions involved in the recombination. In fact, considering vertical or horizontal cuts of the streak image, the trends of the intensity of the emission as a function of time or wavelength, respectively, can be extracted. In this particular example, for InGaAs/GaAs QW, the peak position is measured at 888 nm and the decay time, after a single exponential fitting, is estimated to be 0.7 ns.
Pump-Probe Spectroscopy
In a pump-probe experiment, a pump pulse excites a sample and induces changes that are measured using a subsequent probe pulse. By varying the time between pump and probe pulses, one can retrieve the recovery time scales of the sample. A concrete example is the measurement of the transmittance through a sample. The pump pulse modifies the transmittance of the sample. For minor difference between the pump and probe, the transmittance of the probe will be low and will increase with increasing time delay. By measuring the transmittance as a function of the delay, it is possible to observe the transmittance recovery timescale of the sample. Knowledge of the relaxation of the complete optical gain and index after a sub-picosecond light pulse is essential to assess the potential of current quantum dot materials for photonic systems applications. Pump-probe techniques provide direct, time domain, measurement of gain and refractive index nonlinearities in optical waveguides with sub-picosecond resolution.
Pump-probe spectroscopy is necessary since the timescales of carrier processes in materials (typically tens of pico-seconds) are usually much faster than the bandwidth of conventional detectors. Pump-probe experiments are commonly carried out using picosecond or femtosecond lasers that emit pulse trains. In such a case, the light emitted by a laser is separated into pump and probe beams that reach the sample at different times and, after propagation through the sample, the two beams are again separated in order to analyse only the probe beam. The separation of the pump and probe beams after propagation through the sample can be achieved if the two beams have orthogonal polarisation or different frequencies. This latter solution has led to the development of heterodyne pump-probe spectroscopy.
K. Hall demonstrated the heterodyne pump-probe technique, in which pump and probe pulses are distinguished by inducing a small frequency shift between them, for the first time in the mid 1990’s. The technique allows separate extraction of the gain and refractive index dynamics in the waveguide and works for orthogonally as well as parallel-polarised pump and probe pulses. In the experiment, a high intensity short pump pulse is used to excite the investigated sample by modifying various carrier populations. It leads to changes in the optical properties of the sample, which can be measured as a change of the transmission (gain) or phase (refractive index) of the low intensity short probe pulse.
In order to follow the gain and refractive index recoveries, the delay between pump and probe pulses is changed and the time resolution of the measurement is determined mainly by the duration of the pulses. Both pump and probe pulses follow the reference pulse, which is used for detection purposes. The pump-probe technique provides gain and refractive index recovery at true operating conditions, which are essential for the assessment of the optical devices used in ultra-fast signal processing.
Fig. (right): Animation showing how the gain recovery following the pump pulse (red) is measured by varying the delay between the pump (red) and probe (blue) pulses.
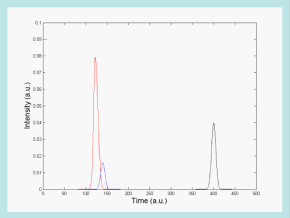
Phase measurement
A change in the amplitude of the reference-probe beating signal, caused by pump-induced changes in the probe transmission, is measured using the R component (magnitude) of the high frequency lock in amplifier. Similarly, a pump-induced change in the refractive index will change the phase of the probe beam with respect to the reference beam. This change is measured directly by the in-phase (X) and out-of-phase (Y) components of the high frequency lock-in amplifier.
In order to perform background free measurements, the pump beam is mechanically chopped at low frequency and the analog output of the high frequency lock-in is connected to a low frequency lock-in amplifier. The low frequency lock-in locks onto this signal, thereby eliminating all changes in the signal, which do not oscillate at the same frequency as the chopper.
Frequency Resolved Electro-Absorption Gating (FREAG)
The Frequency Resolved Electro-Absorption Gating (FREAG) technique gives complete recovery of spectral and temporal information on ultrafast laser pulses in real time, and is similar to, but far more sensitive than, Frequency Resolved Optical Gating (FROG). FREAG pulse analysers work on the same principle as a Second Harmonic Generation (SHG) auto correlator, but use linear transmission through a modulated gate, contrasting with the non-linear crystal required for SHG auto correlators. The result is the same, accurate recovery of real and imaginary parts of the electric field but without requiring high intensity input.
FREAG is very sensitive and can resolve sub-picosecond pulses since it is not limited by the response time of the detector. In addition to this, at each delay position of the gate a complete spectrum is measured, so that both the spectral and temporal characteristics of the pulse are captured simultaneously. Therefore, while a 10Gb/s data stream may look perfectly normal on a conventional oscilloscope, the FREAG will show all the changes in the underlying carrier phase in real-time, allowing complete and rapid optimisation of the system.
Semiconductor mode-locked lasers can spontaneously emit picosecond light pulses at high rates, making them valuable candidates for applications such as optical time division multiplexing. Traditional methods of pulse characterisation such as autocorrelation require high laser intensities and often lose critical pulse information. For example, autocorrelation always returns symmetric traces, regardless of the true pulse shape. With FREAG, a trigger is derived from the laser pulses and used to open and shut an optical gate. The remaining laser light is passed through this gate and a set of laser spectra gathered as a function of pulse delay with respect to the gate. This spectrogram can then be inverted and the full laser intensity and phase information recovered. With this tool, several pulse stabilisation techniques such as dual-tone optical injection have been proven and outstanding pulse jitter performance achieved.
Mode-Locked Lasers
Mode locking of lasers is a means of generating very short pulses, typically on the order of picoseconds or femtoseconds, by enforcing a fixed phase relationship between the many modes of the laser cavity. The laser then only emits when all these modes interfere constructively, resulting in a regular train of ultra-short pulses. Applications for mode-locked lasers include telecommunications (signal encoding), exploration of fast chemical and physical processes, optical data storage, production of THz radiation, and the generation and extraction of clock signals.
There are several different methods for controlling the phase relation between the modes, using either active elements such as acousto-optic or electro-optic modulators, or passive elements such as saturable absorbers. One such arrangement is a two-section monolithic mode-locked laser with InAs/GaAs quantum dot active layer, which consists of a ridge waveguide laser with the ridge, divided into two sections. This allows one section to be forward biased as normal and thus act as the gain section, while the second section can be reverse biased so that it behaves as an absorber. Careful control of the bias voltages determines the saturation level of the absorber section, and can induce mode locking. This approach has the advantage that it is very compact, relatively inexpensive, and easily integrated with existing technologies.
Photonic Sensing
Photonic sensors are key elements in a wide range of applications: security, safety, space, defence, healthcare, multimedia, scientific instruments and others. Photonic sensors provide the means to evaluate samples in-situ obtaining direct molecular specific information through the measurement of rotational-vibrational transitions in the target analytes enabling thus direct qualitative and quantitative analysis without the need to chemically prepare or damage the sample in question. Such sensors are rapid, they can be configured in a portable format and avoid the use of consumables required by most established modern analytical techniques.
Optical sensors thus provide ideal solutions for Process Analytical Technology (PAT) applications as increasingly requested by the chemical and diverse food industries Quantum Photonics. The efficient generation of quantum states of light is a vital task in Quantum Photonics. Current approaches are bulky and expensive with low generation rates and the few commercial single photon sources are either not compatible with telecoms standards, require cryogenic temperatures or are bulky benchtop devices.